By Mara Johnson-Groh
NASA’s Goddard Space Flight Center
Plasma – a fourth state of matter after solid, liquid, and gas where particles have split into charged ions and electrons – is the most common form of matter in the universe. It’s somewhat rare on Earth, but it makes up 99% of the matter in the visible universe. Despite its prevalence, scientists haven’t been able to observationally verify a foundational theory describing how plasma moves in response to electric and magnetic forces. Until now.
With its ultraprecise measurements, NASA’s Magnetospheric Multiscale mission – MMS – has finally measured plasma’s movement on the small scales necessary to see if plasma collectively interacts with electromagnetic fields in the way the fundamental theory predicts, which is described mathematically by the so-called Vlasov equation.
Since the beginning of plasma physics research nearly 100 years ago, the Vlasov equation has often been assumed to be valid for many kinds of plasmas in space. But now the new MMS results, which were published in the journal Nature Physics on July 5, 2021, have allowed scientists to finally see the fundamental plasma variations described in the theory for the first time in nature.
Measuring the basic interactions of space plasmas with electric and magnetic fields helps scientists better understand different mechanisms that fuel energetic space weather events, from auroras to plasma ejections off the Sun, which can interfere with satellites and communications on Earth.
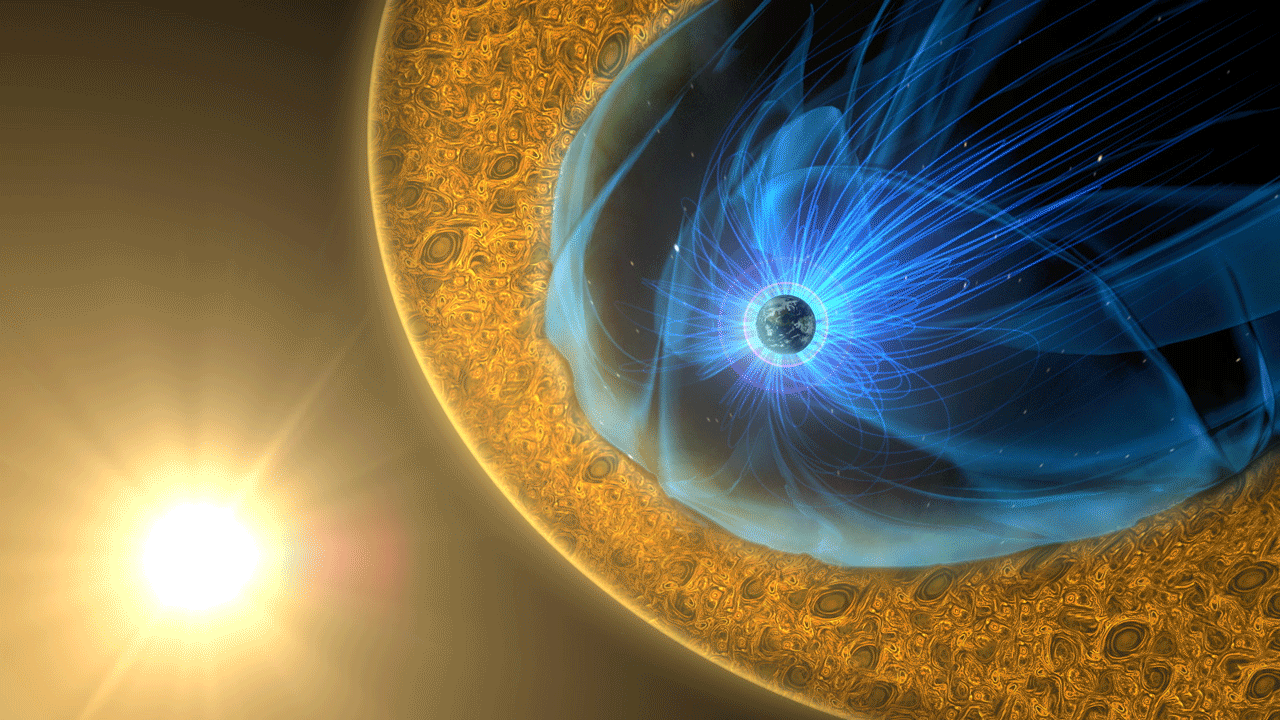
A Century of Plasma Physics Progress
The basic theory of plasma motion originated out of a fundamental theory for gases from the nineteenth century. In the 1890s, an Austrian physicist by the name Ludwig Boltzmann came up with a way to describe the microscopic movement of gases and fluids using statistics. It’s known as the Boltzmann equation, and it’s still taught in physics courses today.
In the 1930s, this work was extended to describe plasmas by Anatoly Vlasov, a Russian physicist. His work specifically described collisionless plasmas, which exist at such high temperatures and low densities that individual plasma particles almost never collide.
Collisionless plasma environments are common in space and can be found in the Sun’s outer atmosphere, solar wind, and in various regions throughout Earth’s magnetic environment, called the magnetosphere.
Unlike ordinary gases, plasmas are electrically charged, as they’re made of positively and negatively charged particles – namely atomic nuclei and their separated electrons. This makes plasmas behave very differently than gases since they are sensitive to electromagnetic forces, which influence their movements. Whereas individual particles in a gas constantly bounce off each other as they erratically travel along, collisionless plasma particles rarely interact and are instead controlled solely by electric and magnetic forces. Vlasov’s equation describes the interplay between plasma particles and electromagnetic fields in these unique collisionless plasma systems – and it has formed the foundation for many ideas about plasmas in the years since.
“The Vlasov equation governs all collisionless plasma phenomena that we know of,” said Jason Shuster, lead author on the new study, Assistant Research Scientist at the University of Maryland in College Park, and MMS scientist at NASA’s Goddard Space Flight Center in Greenbelt, Maryland.
Nevertheless, the terms in the Vlasov equation have never been directly measured because the observation requires seven different types of measurements to be made simultaneously at very small scales in a diffuse plasma – something which is only possible in space.
“In smaller experiments in labs we can’t probe the plasma without disturbing it,” said Barbara Giles, co-author on the new study, Project Scientist for MMS and research scientist at NASA’s Goddard Space Flight Center. “Only in space can we fully immerse instruments within the same phenomenon and accurately test these theories without disturbing the system.”
The MMS Tetrahedron
In 2015, the launch of the four MMS spacecraft changed the way physicists could study plasma in space. Flying in a tetrahedral – or pyramid-shaped – formation with high-resolution instruments, MMS can take measurements far beyond the capabilities of previous spacecraft missions. In their record-breaking tight flying formation, the four MMS spacecraft also fly close enough to measure small-scale properties of plasma, which enabled them to detect variations in terms of the Vlasov equation for the first time.
“With MMS, we can actually probe those minute details at higher resolution than ever before,” Shuster said.
To measure terms in the Vlasov equation, MMS used 64 particle spectrometers – instruments which measure particle energies and charges. The unprecedented spectrometers can simultaneously record multiple types of measurements in position, velocity, and time needed to resolve terms in Vlasov equation. Since each of the four spinning MMS spacecraft has 16 spectrometers sampling particles around its entire circumference, MMS is uniquely able to take these measurements at incredibly high speeds – every 0.03 seconds, which is nearly 100 times faster than previous missions.
“MMS takes advantage of the natural laboratory provided by Earth’s magnetic environment in outer space,” Shuster said. “In the lab, it is very difficult to create a vacuum with a pressure and density low enough to measure the types of electron-scale structures that we’re able to probe with MMS.”
Improving Global Predictions
Now that MMS has observationally confirmed fundamental predictions of the Vlasov equation, this data can be used to better understand phenomena in plasma environments in near-Earth space.
For example, applying small-scale knowledge of plasma to the global-scale magnetosphere can help scientists better understand different mechanisms that fuel energetic space weather events – such as magnetic reconnection, an explosive event unique to plasma that occurs when magnetic field lines sharply reverse direction.
Additionally, just as high- and low-pressure systems create winds and storms on Earth, electric currents and plasma flows drive weather systems in space. Fast moving jets of energetic plasma, such as those scientists observed in this study with MMS, can sustain strong electric currents and pressure gradients which drive space weather phenomena throughout Earth’s magnetic environment.

Having direct observations of the terms in the Vlasov equation provides scientists with a deeper understanding of these basic plasma motions, which enables them to predict the triggers of these fundamental plasma processes more accurately.
“The measurements of terms in the Vlasov equation provide information we can use to constrain and increase the accuracy of our global space weather models, which currently rely on large-scale approximations,” Shuster said. “These discoveries improve our ability to predict space weather operating close to home in Earth’s magnetosphere and deepen our overall understanding of plasmas existing throughout the universe.”